Axial Gradients: Oxygen Pressure Field Theory IV
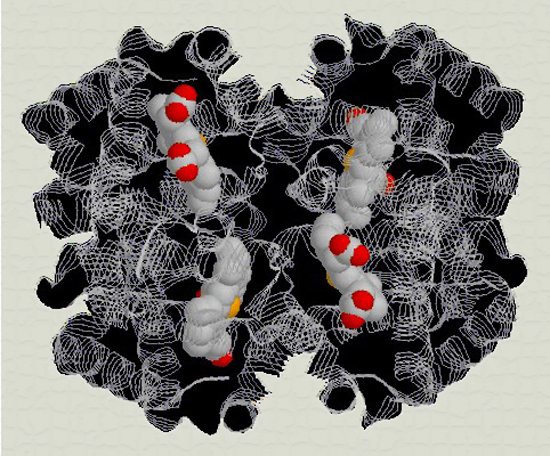
Part IV: Axial Gradients
Authored by: Gary Grist, BS, RN, CCP, LCP
To View all of Gary Grist”s Posts Regarding OPFT- click here
Axial Gradients
To review, Oxygen Pressure Field theory states that there is an oxygen pressure field established in the tissues around each capillary where oxygen is present in varying concentrations from as high as the arterial pO2 to as low as zero. The oxygen pressure field changes as the relationship between the capillary X-section and the Krogh tissue cylinder X-section changes and/ or there is a change in the O2 consumption.
Each capillary is limited in the amount of oxygen it can provide. Increasing intracapillary velocity or carrying capacity of the blood above normal will not significantly improve a capillary’s O2 transfer capability. The increased cardiac output that results from increased O2 consumption provides flow for nutritive capillaries to open. These nutritive capillaries reconfigure the capillary beds from large, deep tissue cylinders to small, shallow tissue cylinders capable of the efficient transfer of large volumes of O2.
The number of these open nutritive capillaries in a given volume of tissue is knows as Perfused Capillary Density (PCD). The body best capable of handling the stress of increased activity or cardiopulmonary deficits is most capable of maintaining adequate PCD. Failure to maintain or improve PCD can result in shock.
Oxygen reaches the tissues where it will be used along two main pathways. The most important of these pathways are the radial vectors. The radial vectors represent the driving force (the capillary-to-tissue gradient) which pushes oxygen from the blood and into the tissue. Radial vectors are perpendicular to the capillary axis and radiate from this axis at right angles in all directions. Under normal, air breathing conditions radial vectors provide 80% of the oxygen reaches the tissues. Any variable which disruptes the radial vectors will result in less O2 being transfered to the tissues. Among these varibles are an increase in the capillary-to-cylinder ratio and a reduction in intracapillary velocity to less than normal speeds (200 microns/sec.).
A second type of pathway by which O2 reaches the tissues is called an axial vector. Radial vectors provide an over-abundance of oxygen to the arterial end of the tissue cylinder , while providing just enough oxygen at the venous end to prevent the formation of a lethal corner. This sets-up an O2 gradient between the arterial-end tissues and the venous-end tissues. This gradient runs outside the capillary but parallel to its axis.
Thus the Term Axial Vectors.
(Click Image above to View PowerPoint Presentation)
In our Krogh model (see the figure file Krogh.bmp or ..PCX in Part 1), the arterial-end pO2 averages 20 mmHg. The venous-end pO2 averages 10 mmHg. Therefor, the axial gradient is only 10 mmHg on average. However, this is enough to push significant amonts of oxygen from the arterial end to the venous end. The axial vectors have a much greater distance to traverse. The axial vectors move from the arterial-end tissues to the venous-end tissues, an average distance of 200 microns. The length of capillaries vary from 100 to 300 microns, with 200 microns being the average.
Despite this large distance, axial vectors provide about 20% of the oxygen to the venous-end of the tissue cylinder under normal conditions. Under times of stress, axial vectors can pick-up some of the slack caused by a reduction in the magnitude of the radial vectors near the venous end of the cylinder. For example, as intracapillary velocity falls the radial vectors near the venous end of the cylinder decrease as a result of desaturation of the Hg (the SVO2 falls). This causes a drop in the average venous-end tissues pO2.
In this example, the venous tissue pO2 drops from 10 mmHg to 5 mmHg with a lethal corner begins to form. However, the arterial-end tissues are still well oxygenated with an average tissue pO2 of 20 mmHg. The axial gradient increases from 10 mmHg in the earlier model to 15 mmHg. This represents a 50% increase in the axial driving gradient and will increase the supply to the venous-end tissues from the previous 20% to 30% of the total. It has been estimated that as intracapillary velocity drops to 50 micons/sec., axial gradients provide up to 80% of the oxygen reaching the venous-end tissues.
Knowing how axial vectors augment venous-end tissue oxygenation makes it possible to understand how very high arterial pO2 values can be of importance. Even though an arterial pO2 over 150 mmHg does not contribute significantly to oxygen carrying capacity of the blood, it causes a redistribution of oxygen from the saturated arterial-end tissues along axial vectors to the venous-end tissues. In our model of a 1/4 Krogh cylinder an arterial pO2 of 500 mmHg would raise the average arterial-end tissue concentration from 20 mmHg to about 125 mmHg. If the venous-end tissue concentration is 10 mmHg the axial gradient would be 115 mmHg or 11 1/2 times normal.High arterial pO2 values that increase axial vectors to improve venous-end oxygenation is called “axial kick”.
In the worst case scenario where a lethal corner forms as a result of poor perfused capillary density AND reduced intracapillary blood flow velocity, axial vectors may be supplying oxygen to the majority of the tissue cylinder. However, this patient receives only a short repreive on 100% oxygen. Even if axial kick adequately supplies O2 to the distal cylinder, waste products, mainly CO2, will accumulate in the lethal corner area. The accumulation occurs because the tissue cylinder is too large for the capillary supplying it and the slow intracapillary velocity affords poor removal of CO2 from the tissues. The result is a intracellular pH change so extreme that normal cell function cannot proceed despite the presence of oxygen. This predicament will be discussed in a future posting.
Addendum:
The Fick formula notwithstanding, axial kick might be more important than oxygen delivery in some situations. As discussed earlier in Part 1, the perfusionist may be able to estimate the tissue oxygen supply and safe arrest time prior to a deep hypothermic and circulatory arrest. If the perfusionist uses a low oxygenator FiO2 to keep the patient’s arterial pO2 low prior to arrest (150 mmHg or less), it won’t matter how much oxygen is being delivered in terms of cc/min.
If the radial and axial vectors are not increased, no significant amount of oxygen will be stored in the cold tissue cylinders. If all the dissolved oxygen in the tissues is consumed after 30 minutes into a 60 minute arrest, then tissue hypoxia and anoxia will occur, albeit at a relatively slow rate. Reoxygenation may then result in the dread reperfusion injury. If the perfusionist utilizes the radial and axial vectors to their maximum capacity to fully saturate cold tissue cylinders prior to arrest, reperfusion might be avoided.
During the arrest period, although the radial vectors would not be present, axial vectors would continue to redistribute oxygen during the arrest phase because they are not dependent on capillary blood flow. If oxygen storage is adequate and the arrest period ends before oxygen stored in the tissues is used up, then reperfusion injury could be avoided. Reperfusion injury only occurs after a period of hypoxia or anoxia.
If you can avoid hypoxia, you avoid reperfusion. A problem remains, however. During the arrest, without blood flow, CO2 builds ups intracellularly changing intracellular pH. This may be the primary limitation for a deep hypothermic arrest, not lack of oxygen.